Introduction
My goal was to see what a starship would look like if designed and built this century. This meant I would only use current or those technologies that with a decade or two of reasonable extrapolation, would be available. In the first article I laid out the challenges and some of the limitations that will constrain our design by reviewing the rocket equation and gravitational slingshots. With the rocket equation, I limited our design to a Mass Ratio (MR) of 20 since this is both technologically feasible and provides for an acceptable velocity three times greater than the exhaust velocity.
Using a single stage chemical rocket with a specific impulse of 460 would give us a final velocity of around 13kps. Using Nuclear Engines with a specific impulse of 1000 could get up to 30kps. At this velocity it would take about 45,000 years to get to the nearest star… clearly too long.
Electric propulsion, in the form of ion or plasma engines have a much higher performance than chemical or nuclear. Ones that are currently in use have specific impulses of 3000-4000 seconds. It is anticipated that with enough power electric thrusters could reach 10,000. However, there are two challenges with high specific impulse engines- their thrust is extremely low and they need a lot of power. The low thrust means that they may take centuries to exit the solar system and achieve escape velocity and that they may take thousands of years to accelerate to our target speed.
Problem Scope
Two items are needed to leverage the high performance of electric thrusters – a first stage that is energetic enough to get the second stage with its electric thruster to a solar system escape velocity, and a second stage electric thruster that has a very high power source but low mass.
Solution – Two Stages- Achieving Solar System Escape Velocity
The partial solution to this issue is to develop a two-stage rocket with the first stage having a high thrust chemical or nuclear rocket and combining it with an Oberth Powered Maneuver which will allow our starship to reach a moderate escape velocity quickly. Once this stage is done, it will be discarded, and the electric propulsion will kick in and add to this velocity.
The Oberth Powered Maneuver.
The Oberth or Powered Maneuver is an effective option to substantially increase our starships velocity. It is done by performing a dV rocket burn near a planet or star. The velocity provided by a powered maneuver is described by the equation.
EQUATION 0-1: | ![]() |
Where:
V= velocity after the powered maneuver
Δv = delta v of burn at periapsis
Vesc = escape velocity at periapsis
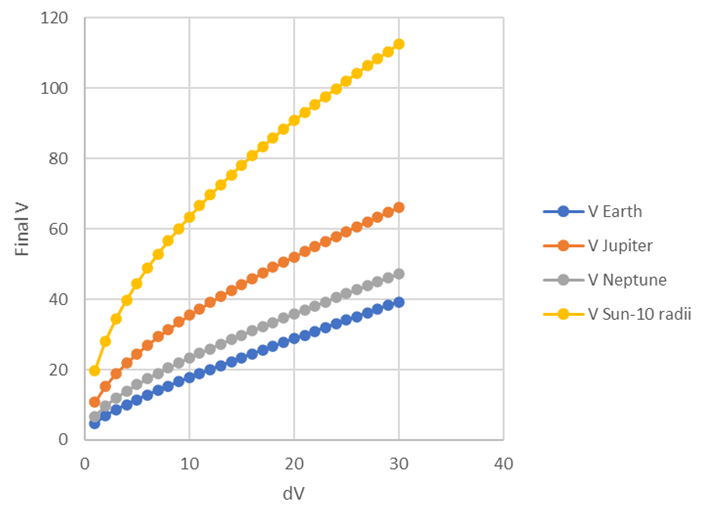
FIGURE 0-1: OBERTH POWERED MANEUVER (NOTE THAT IN THESE CALCULATIONS THE PERIAPSIS DISTANCE FROM THE CENTER OF THE BODY WAS- EARTH- 7000KM; NEPTUNE 28,000KM; JUPITER 75,600KM; SUN 6,757,000KM).
Periapsis is the closest point of our approach to the planet or star. One requirement of a powered maneuver is that to maximize the velocity increase we need to apply our dV as quickly as possible – the quicker the better. For a close dive near a planet, this time will usually be measured in minutes- 15 minutes being a good target. For around a star like the sun, because we can’t get as close as we can to a planet (due to the radiation and associated heat as well as its bloated diameter) as well as the fact that the gravitational field is so much larger, we can increase our dV burn time to a couple of hours with a minimal impact to the ideal velocity change. Our engines must be powerful enough and our spacecraft light enough so that the dV can be applied in this short time frame. This means that for a powered maneuver, we are limited to either a high thrust Nuclear Thermal or a chemical rocket. Nuclear Thermal rockets have been an area of active research and development for 70 years and have demonstrated Specific Impulses in the 850sec plus range. It is believed that improved derivatives of these engines may be able to have a specific impulse of 1,000 seconds, or a little over twice that of the LH/LOX SSME derivative.
Using a powered maneuver with a dV of 10kps and a close Solar approach to within 10 solar radii would leave us with an impressive velocity of a little over 60kps. At this rate we could reach the nearest star in “only” 22,000 years or so. A voyage this long would indicate a large, crewed starship – it is unlikely that a small uncrewed starship could be built that lasts this long. Extensive maintenance as well as hundreds of nuclear fuel changeouts would be required. Despite 60kps being extremely fast (about 6x greater than our original Saturn V capabilities), we would desire to go much faster.
With a two-stage rocket, the first stage would perform a powered maneuver around the sun, and the second stage would continue to accelerate with a low thrust electric propulsion engine. I considered several versions of a two-stage rocket, and settled on one where each stage had a MR of 10. The highest performance obtained with a reasonable payload and structure mass used a Nuclear Thermal (with an specific impulse of 1000 sec) for a first stage powered maneuver around the sun at 10 radii, followed by an electric propulsion engine of 1000 seconds specific impulse. This combination gives us an impressive speed of 300kps. A starship with this performance would be able to get to the nearest star in a bit more than 4300 years, and approach 10 light years in 10,000. With current technology, this is likely the highest feasible velocity.
Even though 300kps is an impressive achievement the two stage rockets leaves several open issues that need to be addressed. The biggest are:
- Mass of our power supply. Our power supply may consume all of our available m1 mass and may not provide enough power to effectively accelerate our starship.
- We want to stop at the target star which means we also have to decelerate from 300kps. With a specific impulse of 10,000 seconds this means we need to increase our starting mass by at least another 20x in order to have mass to decelerate. This will make our m0 before the sun centered powered maneuver so large that it will make it impossible to achieve the 10kps target for our powered maneuver.
- Since our voyage will be at least 4300 years we need a large vessel baseline starship (m1). From this we can establish our m0. We will need to determine a reasonable m1 before we can determine what our performance requirements (and power requirements) for our propulsion are.
These problems cannot be solved easily- several solutions will be needed.
Solution – Lightweight but High-Power Reactors
The first item to address is designing a lightweight but high power reactor. To date, spacecraft have primarily counted on solar panels or Radioisotope Power Generators (RTG’s) to provide power. For an interstellar mission, some form of nuclear power is required but RTG’s, while reliable, are very inefficient- converting less than 7% of their thermal heat to electric. The typical RTG provides 4.2we/kg of reactor mass. Furthermore, RTG’s typically use plutonium, an artificially created material that is very expensive and highly regulated (due to its ability to make nuclear weapons). No RTG missions have ever created more than about 1000watts of electricity. For larger electric propulsion engines 100kW of power or more are typical. If we had enough plutonium (which we don’t) a 100kW electric thrust engine would mass about 29mt.
For a high-power mission fission power is a more viable solution. Fission plants, like those on earth, can generate vast amounts of power and use naturally occurring Uranium 235. The problem is that no fission reactor has ever been built and used in space. The Kilopower Reactor Using Stirling Technology (KRUSTY) was a NASA proposed space nuclear reactor that was to develop 10kw of power from about 44kg of highly enriched Uranium. Its design goal was to generate 6.67We/kg or, if converted to Specific Mass as shown in the Figure 0-4, 150kg/kWe. A smaller demonstration model was successfully tested in 2017.
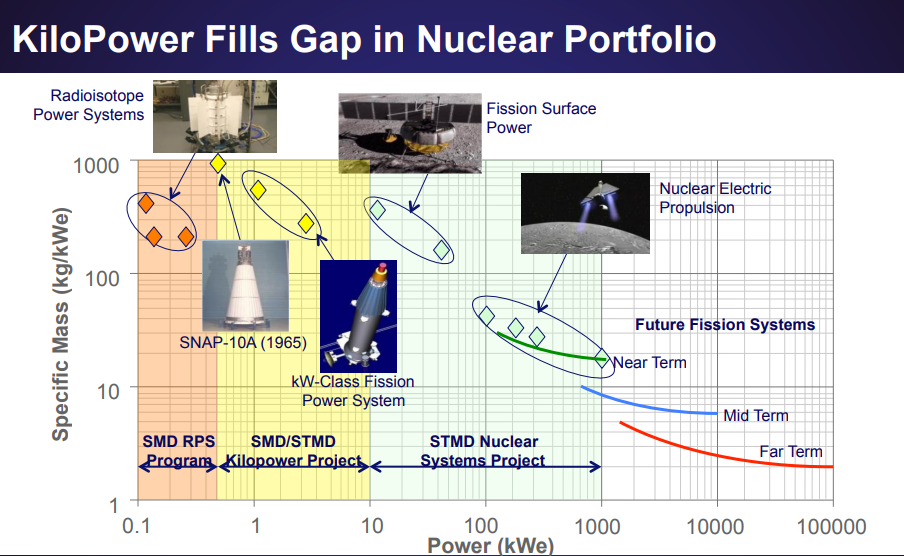
FIGURE 0-1: Nuclear Technology: KiloPower Fills Gap in Nuclear Portfolio (Source: NASA)
How large a thrust is needed is determined by how massive our starship is, so to calculate the minimal acceptable Specific Mass for our power system that will enable our mission is an iterative process where the variables are adjusted. By running various scenarios, I determined that the bare minimum requirement is a power plant at least 3x more mass efficient than the KRUSTY design, or 20We/kg or a Specific Mass of 50kg/kWe. If our power plant puts out less than this, we quickly wind up dedicating all our spacecraft m1 mass to the power plant and have nothing available for anything else (habitation module, fuel tanks) etc. If we attempt to limit the mass of our reactor to leave room for other equipment as well as our reaction mass tank, our acceleration becomes so low that it takes thousands of years to get up to full speed.
Requirement – How big does our ship need to be for a 4,300 year mission?
The effectiveness and applicability of the above proposed solutions depend to a great extent on how big our starship will be. A starship that starts out as a two-stage vessel but counts on a powered maneuver may have an m1Stage1 mass that is so large the ship will need an unreasonable amount of engines and unrealistically large amount of reaction mass to perform the maneuver in our short timeframe.
Size and Mass of Crew Tori
Since our voyage will last thousands of years a crewed mission needs to be viable for hundreds of generations. There have been many studies that have attempted to determine the minimal size needed to have a multi-generational population. These studies have indicated anywhere from a few dozen to ten’s of thousands. I decided to pick a crew size of 1000. This size, combined with frozen embryo’s and sperm should ensure sufficient genetic diversity and enough resources to maintain the ship. I then looked at how large the ship would need to be to support this population for hundreds of generations.
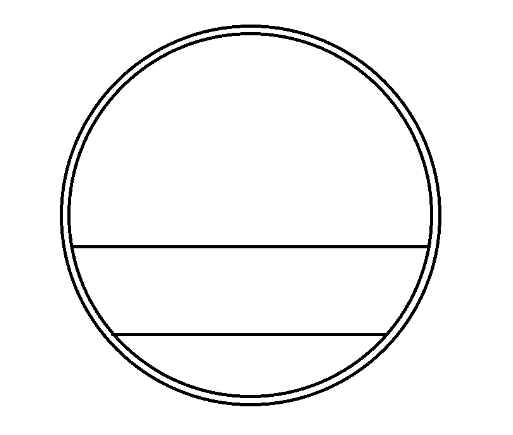
Figure 0-1 Torus Cross Section
In the 1970’s Gerard K O’Neil pulled together a team to develop a space settlement plan (Johnson & Holbrow, 1977). In this study, it was estimated that about 10 acres of intensively cultivated land could support every 1000 people (Heppenheimer, 1977, p. 128). I believe that with genetically modified organisms, hydroponics, and additional improvements in farming including growing of artificial meat (eliminating the need for cows, pigs, etc.) we may be able to double this productivity. Using this rule of thumb, we will need 20,000 m2 of land which equates to about 5acres dedicated to farming/food to feed the colonists. Some additional area would need to be set aside for housing, offices and manufacturing.
The same Space Settlement study also determined that the most efficient structure for a small or medium sized colony was the torus. Using a double deck configuration (Figure 0-7), and adding some land for offices, public spaces, and apartments, the minimal size that would be viable is one with a minor diameter of 15m which gives almost 28,000m2 on two decks. I used a major diameter of 204 meters to give a gravity of .9 earth normal at 2 rpm. By calculating the mass of the structure including deadweight, we could build a torus structure of about 17,000mt if made of aluminum. Adding additional mass as a margin of error and including water and soil for crops 19,000 mt would be more likely. For stability, a second counter rotating torus would be added to bring the total mass of the two tori to 38,000mt. One of the tori would be for habitation and include farm land. The second torus would serve as storage for supplies as well as contain some of our power (reactors).
Mass of Cosmic Ray Protection
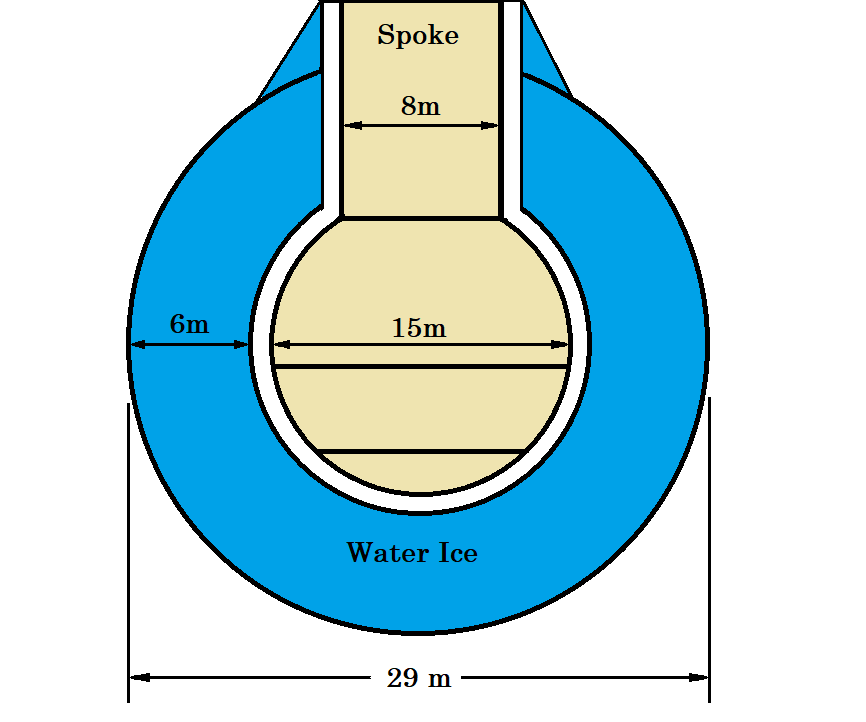
Figure 0-2 Cross Section of Torus with Cosmic Ray Protection
One striking aspect about building a starship is the amount of mass needed for passive cosmic ray protection- this turns out to exceed by far our tori, powerplants, and MD. Literature indicates that the best materials are polyethylene or water from 6 to 7 meters thick (Globus). It turns out that to wrap a single torus with a minor diameter of 15m with 6meters of water (with a one-meter standoff distance) would mass about 550.000mt. If we use this amount of mass for our starship during our powered maneuver our mass is too large to perform a 10kps dV in the short amount of time we have allocated.
I targeted a 10kps dV maneuver during a 2-hour closest approach to the sun. Using Nuclear Engines with a specific impulse of 1000seconds we would start out with a mass (m0Stage1) around 582,000mt and end with a mass of 210,000mt. If we elected to use a LH/LOX engine similar to the SSME our m0 would be very large- just shy of 2 million mt- difficult but not impossible- we would need the equivalent of 116 SSME engines firing for two hours. It is hard to see how we could construct a much larger ship that is able to perform a dV so quickly. What this means is that our starship would have to have minimal cosmic ray protection- clearly not safe for a 4000+ year mission.
In addition to our tori massing about 38,000mt, and our cosmic ray protection as being as high as 550,000mt we would need additional mass for our power supply and engines. If we assume that engines with a 10,000 second specific impulse, a velocity of 300kps and a ending mass m1Ending of 200,000mt we will need a 4 million mt m0 in order to stop at the target star. This is far to much mass to perform our powered maneuver or, if strictly using electric thrusters, to reach our target speed in anything less than tens of thousands of years. The bottom line is that we cannot make a starship work.
Areas for Engineering Improvements
We determined that so as not to use up all our mass for our power plant, we will need a power plant that generates at least 20W/kg. Any improvement to this will permit either a faster acceleration or allow for more mass be allocated for other purposes.
Conclusion
We currently have a starship with the following specifications:
- A two-stage configuration, the first a high thrust engine to perform a powered maneuver to reach a solar system escape velocity, and the second a lower thrust mass driver.
- A 300kps top speed
- An m1 mass of about 200,000 mt for a crew of 1000
With these characteristics, our starship would still require a voyage time of over 4300 years.
Unfortunately, this would be a very poor starship. Its problems would be:
- Inability to stop. Using a specific impulse of 10000, a velocity of 300kps we would need a starship of 4 million mt but after our powered maneuver our mass was only about 200,000mt.
- Even at 200,000mt our ship is too small. We have:
- Inadequate Cosmic Ray Protection. We only know our torus mass is about 38,000 and our cosmic ray requirements are about 550,000mt.
- We have not calculated the power supply and our engine mass. Furthermore, we have not addressed our starships need for power and the replacement of lost materials that will be inevitable over a 4000+ year mission
In the next article we will discuss packet resupply which can address both of these issues.
A more complete assessment of the challenges of building a starship not addressed in this series of articles are discussed on my website www.allthingsspace.info.
References
- Globus, A. (2017) Orbital Space Settlement Radiation Shielding. NSS Journal of Space Settlement
- Heppenheimer, T. A. (1977), Colonies in Space. Harrisburg, Stackpole Books
- Johnson, Richard D, Holbrow.(1977), Space Settlements: A Design Study. Washington DC: Scientific and Technical Information Office, National Aeronautics and Space Administration
About the Author: David W McGuire is a retired veteran from the USAF and AF Reserve where he held various jobs as an Acquisition and Procurement Officer, C-5 Maintenance Officer, Maintenance Squadron Commander and Deputy Group Commander. He retired as a Colonel. He also has worked in the chemical, pipeline and petroleum industries where he held varied positions including Terminal Manager, Regional Accountant Manager, Regional Engineering Manager, and Regional Operations Director. He has a degree in Aerospace Engineering and an MBA.